Quantum biology continues to intrigue
What if you were told that a single proton or electron can influence the behavior of an entire biological molecule? Would you snort with derision or give the idea some serious thought?
The loosely defined field of quantum biology, which is permeated in equal parts by speculation, skepticism and unbridled excitement, looks to that strange realm of physics called quantum mechanics where energy starts acting funny. Researchers working in this niche are asking if some critical biochemical reactions rely on the split personality of energy as waves and particles.
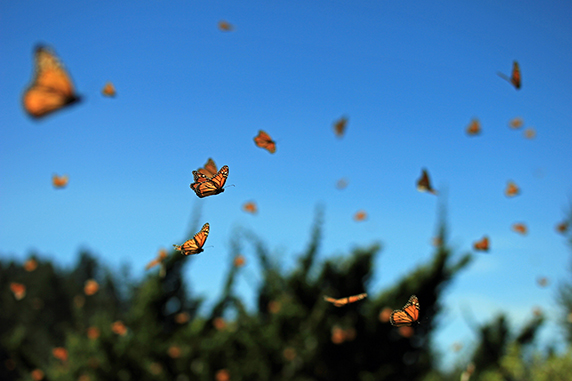
Superficially speaking, quantum mechanics pervades all chemical reactions including ones in the specialized collection called life. “If you delve into chemistry deep enough, you inevitably come across quantum mechanics, in the sense that the orbital structure of atoms is based on quantum mechanics,” says Johnjoe McFadden at the University of Surrey in the U.K. So, in a sense, he adds, “quantum mechanics is everywhere.”
Quantum effects tend to fade at the level of whole atoms and molecules. At this level, classical mechanics, the realm of forces laid out by Isaac Newton and others, begins to dictate the movements of objects. Quantum mechanics also is thought to be most noticeable at temperatures close to absolute zero. The general thinking goes that there is no way quantum effects could persist at the relatively balmy temperatures of life.
But what if — just what if — they did?
A pressing problem with the field of quantum biology is the inability to test and prove unequivocally many of the ideas within its purview. For that reason, even the most ardent supporters of quantum biology are its biggest skeptics. “I’m always the biggest critic as well as a fan,” says Greg Scholes of Princeton University, who is studying quantum effects in photosynthesis.
Jim Al-Khalili at the University of Surrey, who has gone as far as giving a TED talk and co-writing a book with McFadden on quantum biology called “ Life on the Edge,” says that the field needs to be treated with caution. “We have to be skeptical,” he says.
Quantum mechanics brushes against genetics
The concept of quantum biology first arose in the late 1920s. Quantum physics was in its heyday after the discovery that particles existed as discrete packets of energy that could act like waves. The word “quantum” refers to those wave-particle packets of energy.
When quantum physicists established the mathematical basis for quantum mechanics in the early 20th century, they “strode out of their labs around Europe, arrogantly looking around for other problems to solve,” says Al-Khalili. “The biologists looked like they needed some help — they couldn’t understand what a gene was.”
At this time, biologists were wrestling with understanding what a gene was made of, how it functioned and how it propagated from one generation to the next. It was natural for scientists to wonder if the underpinning of genetics at the atomic level could be explained by quantum phenomena. After all, certain areas of physics and chemistry, such as condensed-matter physics and computational chemistry, make sense only in view of quantum mechanics. Why would biology be different?
Physicists who pondered the atomic and molecular processes of life included Max Delbrück and Erwin Schrödinger. Delbrück was an author on a paper that considered the effects of ionizing radiation on genetic matter. That paper, “On the nature of gene mutation and gene structure,” inspired Schrödinger’s book “What is Life?” The book devoted much attention to quantum effects in biology, with Schrödinger postulating how order at the level of the hereditary material could lead to order on the organismal level.
“What is Life?” is said to have motivated James Watson and Francis Crick to think about the structure of DNA. In 1953, Watson and Crick published the double-helix structure. “What came thereafter was that there was this strong sense that quantum mechanics was no longer needed in biology,” says Al-Khalili. “Molecular biology was doing very well without quantum mechanics, thank you very much.”
The notion that quantum effects may play important roles in molecular biology went out of fashion. But beginning in the 1970s, quantum effects started to be considered again in disparate subject areas: Do quantum phenomena occur when some species of birds migrate? Are they present during the first stages of photosynthesis? Do they influence enzyme catalysis?
Biological relevance
What makes quantum biology of today different from the science of yesteryear is that researchers are designing experiments and developing theoretical models based on experimental data. As Alexandra Olaya–Castro at the University College London says, quantum biology is “now driven by experiments.”
But hesitation abounds in calling quantum biology an actual field of study, even among the scientists who work on quantum phenomena in various biological processes. “I’m not sure about calling it quantum biology. It’s clearly in vogue at the moment,” says Peter Hore at the University of Oxford in the U.K., who is interested in understanding the molecular mechanism by which migratory birds sense Earth’s magnetic field. “It’s an attractive idea that nature has adopted, and optimized, fundamentally quantum phenomena for its own purposes.” But, he adds, “my sense is that it has really not (been) established yet that any of these phenomena are genuinely quantum mechanical.”
Much of the skepticism is rooted in the fact that quantum mechanical experiments are hard to do. Typical experimental conditions in quantum mechanics are near absolute zero, under vacuum and vibrationally isolated — hardly physiological. Biochemical analyses are another ball of wax. Measuring quantum phenomena in molecular biology can be an artful arrangement of experimental compromises. But then the problem becomes proving that the quantum effects being measured in experimental systems actually happen in the real world. It’s challenging to show unambiguously quantum effects in biological experiments.
And then comes the question of relevance — are quantum phenomena making a difference in biochemical processes, or can they simply occur without having any meaningful effects? The very fact that most molecular biologists and biochemists don’t contemplate quantum mechanics in their line of work says something. “Right now, biologists don’t care” about quantum mechanics, says Scholes. “We have to listen to that. It means we haven’t proven biological relevance.”
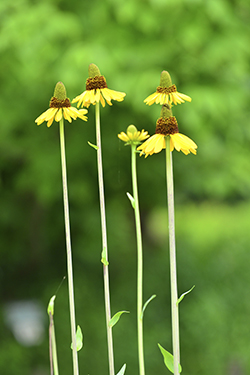
The poster children
So far, researchers have demonstrated quantum effects most clearly in enzyme catalysis and photosynthesis. Both areas are most advanced in their experimental data and theoretical underpinnings. Another line of work is avian migration; the centuries-old question of how migratory birds navigate from one part of the globe to another continues to dog researchers.
Enzymes.Judith Klinman at the University of California, Berkeley, has been studying quantum effects in enzyme catalysis since the late 1980s. She seems surprised that her body of work is held up as one of the prime examples of quantum biology. “I never thought it would be called a field,” she says.
Over the years, Klinman’s group and collaborators have shown that hydrogen tunneling occurs in enzymes. The fact that electrons can tunnel, which means to cut across an energy barrier instead of going over it, is undisputed. Hydrogen tunneling is another matter. Hydrogen is 2,000 times heavier than an electron. It wasn’t clear, when Klinman and her team began looking at isotope effects in enzymatic reactions in the late 1980s, that this heavy entity had that requisite quantum duality of wave and particle.
But in doing the kinetic isotopic effect experiments with enzymes such as alcohol dehydrogenase and soybean lipoxygenase for more than two decades, Klinman and colleagues showed that hydrogen tunneling was occurring in biological molecules at room temperature. The experiments involved replacing hydrogen with its heavier isotopic counterpart deuterium and measuring how the catalytic pace of the enzyme changed.
Initially, Klinman says, the data suggested that a simple tweak to an existing theory based on semiclassical mechanics would explain what was going on. But as more data accumulated, it became obvious the tweak wouldn’t suffice. A new explanation was needed. “I didn’t really believe it at first,” says Klinman, recalling her reaction when she first had to consider that quantum mechanical behavior was occurring.
That new explanation was that a hydrogen, like an electron, was cutting through an energy barrier rather than going over the hump. New data suggest motions of the enzyme are critical for the quantum phenomenon to occur. The motions of the enzyme bring two sites, the acceptor and donor that are needed for the reaction to take place, very close together — so close that the hydrogen can move like a wave to get from one site to another.
The emerging picture is a “ very different view of catalysis,” says Klinman. “The role of the whole protein, through these fluctuating conformations, is to bring things so close that quantum mechanics starts to take over, even at room temperature.”
Photosynthesis. A longstanding question in biology is how the energy from sunlight gets transferred through chlorophyll molecules to the photosynthetic reaction centers with nearly 100 percent efficiency and within picoseconds.
In 2007, Graham Fleming’s group at Berkeley published a paper that suggested a role for quantum mechanics in photosynthesis. They described their analysis of the Fenna–Matthews–Olson complex. The FMO complex appears in green sulfur bacteria that live in the Black Sea and other sulfide-rich waters. The FMO complex contains a type of chlorophyll, a class of molecules that can absorb photons and transfer the electronic excitations, which are created during the absorption process, to the reaction center of the photosynthetic apparatus. These electronic excitations are quantum mechanical entities called excitons.
The investigators used an ultrafast spectroscopic technique that allowed them to study the complex. In analyzing the data, “we saw a long-lived oscillatory signal that was lasting far longer than we would have expected,” says Greg Engel, who was the first author on the paper as a postdoctoral fellow working with Fleming. “It was lasting, interestingly, on the timescale of the energy transfer times in these complexes.”
The collective electronic excitations of interacting chlorophylls were thought to cause the oscillatory signal. How the oscillatory signal came about became a pressing question in the field. But after years of debate, says Olaya-Castro, a quantum phenomenon was the answer. The data suggested that the energy was traveling by multiple routes in the chlorophyll molecules simultaneously, all within a couple of picoseconds.
McFadden uses a traffic jam to explain the quantum phenomenon: “You can go one way or another way to escape the traffic jam. But you never know, once you’ve taken one route, whether the other route would have been better. But for the energy in photosynthesis, it travels through all routes and then crashes down on the one that works out the best.”
A criticism of the 2007 observation was that the isolated FMO complexes were analyzed at -196 °C, a temperature far from being physiologically relevant. In 2009, the group of Ian Mercer at University College Dublin reported similar observations at ambient temperatures with the light-harvesting complex II of a photosynthetic bacterium. In 2010, Scholes’ group demonstrated the phenomenon in aquatic algae, and Engel, now an independent investigator at the University of Chicago, repeated the FMO experiments at higher temperatures. The observation has been extended beyond bacteria and algae to protein complexes in spinach.
As more research has gone into the mechanism of excitation transfer within chlorophyll molecules, it’s becoming apparent, as it has for enzyme catalysis, that specific motions of the pigment are critical for allowing the quantum phenomenon to occur. “Normally in biology, you focus on the paradigm of structure–function, but these observations are adding an element to that paradigm that should be structure–dynamics–function,” notes Olaya-Castro.
However, researchers still feel they are not getting a proper look at the mechanism of excitation transport. “What we need is to develop new kinds of measurements,” says Scholes. The kind of new measurements he’s thinking of will allow researchers actually to see the energy moving through the chlorophyll molecules to the reaction centers.
But that’s easier said than done. “We lose so much information as complexity scales,” says Scholes. “That’s one of the issues here. We can do these pretty deep analyses and incisive experiments on these isolated light-harvesting complexes, but what happens when you have 100 of them working together in an organism which has other things to worry about as well?”
Avian magnetoreception. Before the onset of winter every year, European robins make their way from Scandinavia to the Mediterranean or North Africa. Since the 19th century, zoologists have wondered if migratory birds follow Earth’s magnetic field. It wasn’t until 1976, with work done by the husband–wife team of Wolfgang and Roswitha Wiltschko, that the answer became obvious. Yes, birds like the European robins navigate by magnetoreception, which means by sensing Earth’s magnetic field. But now the question was how.
In 2000, Klaus Schulten’s group at the University of Illinois Urbana–Champaign proposed that magnetoreception relied on cryptochromes, a class of molecules ubiquitous in many species, as the internal compass in birds.
Cryptochromes are found in the eyes of most animals. Although cryptochromes are implicated in regulating circadian rhythms, Schulten’s group put forward the idea that cryptochromes play a role in photosensitive magnetoreception. Inside the cryptochrome, the authors suggested, there was a coupling between unpaired electron and nuclear spins. The coupling is a quantum phenomenon known as entanglement.
The proposal had a historical context. Back in the 1970s, Schulten originally had suggested that, in the presence of light, unpaired spins coupled in a way that was sensitive to a magnetic field. The 2000 paper by Schulten’s group pinpointed the spins to cryptochromes, which “gave us all a specific molecule to think about,” says Hore. (In their book, Al-Khalili and McFadden call that paper “one of the classic papers of quantum biology.”)
Since then, there are some hints that cryptochromes in fruit flies and monarch butterflies (like European robins, monarch butterflies also migrate) are sensitive to a magnetic field. For example, in 2008, Steven Reppert’s group at the University of Massachusetts published a paper demonstrating that fruit flies missing cryptochromes were insensitive to magnetic field effects.
The question now dogging researchers studying avian magnetoreception is how a single molecule sitting in an animal’s eye can sense Earth’s very weak magnetic field, do some quantum mechanics and set the animal in the right direction. “Those are challenging experiments to do,” notes Hore, whose group has being working in the area.
Just on the molecular-biology front, “we have no idea about what the environment of these proteins might be inside the cell,” says Hore. “We really have no idea about what the binding partners are of the cryptochromes in the context of magnetic sensing. It’s almost certain the proteins will have to be immobilized to stop them rotating inside the cell, because if they do rotate then they won’t be able to sense the rotation of the magnetic field, merely its presence. That’s not enough for a compass.”
Then come the issues of proving that the phenomenon actually happens. All molecules possess thermal energy simply because of their random motions. This energy may override weak magnetic effects. Skeptics have pointed out that because the Earth’s magnetic field is so weak (refrigerator magnets are stronger than Earth’s magnetic field), any interactions of a molecule with the magnetic field will get swamped by the molecule’s thermal effects.
The answer to the skeptics is timing. “If you manage to do the process fast, before the thermal effects take over, that thermal energy becomes irrelevant,” says Schulten.
And then comes the task of proving biological relevance. In nonbiological molecules, quantum entanglement is known to happen. “But the entanglement doesn’t give you anything extra,” says Hore. The entanglement simply exists. The same may apply to radical pair spins in biological molecules; the quantum entanglement may not exert any effects.

Be skeptical, not dismissive
There are more ideas in quantum biology. Olfaction, the science of how we smell, has been linked to quantum effects. So has consciousness, with proposals that quantum processing of atomic spins may be at the core of consciousness or that voltage-gated ion channels may act as the centers for quantum effects. These areas are surrounded by more skepticism and controversy than the other areas of quantum biology.
Al-Khalili and McFadden are studying whether hydrogen tunneling can cause DNA mutations. The idea goes back to the Swedish physicist Per-Olov Lowdin, who proposed in 1963 that hydrogen tunneling occurs between the paired bases of A, T, G and C, creating tautomers of the nucleotides that then mismatch during DNA replication.
But the experiments, which McFadden’s group is now planning, are difficult to interpret. The theoretical modeling by Al-Khalili’s group so far has shown that the amount of hydrogen tunneling occurring in DNA is so rare that it’s insignificant. Although Al-Khalili’s group is going back to the drawing board to try other theoretical frameworks, “if your results say hydrogen doesn’t tunnel across DNA strands, then it doesn’t,” says Al-Khalili. “You have to live with it. You can’t just make stuff up. That’s where we are at the moment.”
The one thing experts unanimously say is that they need new technologies and methods to delve deeply into the questions being asked about quantum effects in biology. Without having the experiments that unequivocally show that quantum effects are taking place in molecular biology, Olaya–Castro says the field will remain hung up on the question, “Are you sure you’re seeing quantum effects?”
“We need to move this field forward to actually say for sure, ‘Yes, here is the proof that it is quantum,’ so we can move on now to address what is, I think, the most important question: ‘What advantage does this bring to biology?’” she says.
But until that happens, the field will be hounded by skepticism. However, skepticism, which the experts welcome because they say it makes for better science, isn’t the same thing as outright dismissal. Some experts say their ideas get rejected outright by biologists who have built entire careers without having to ponder the vagaries of the quantum world.
“Quantum mechanics is required to explain so much that underpins physics and chemistry. It’s not beyond the bounds of possibility that it can underpin, in a very real and nontrivial way, certain mechanisms and phenomena in biology,” says Al-Khalili. “But just because you’ve not had to learn quantum mechanics and have the headaches of figuring out how a particle can be in two places at once, it doesn’t mean quantum mechanics doesn’t happen in biology.”
Enjoy reading ASBMB Today?
Become a member to receive the print edition four times a year and the digital edition monthly.
Learn moreGet the latest from ASBMB Today
Enter your email address, and we’ll send you a weekly email with recent articles, interviews and more.
Latest in Science
Science highlights or most popular articles
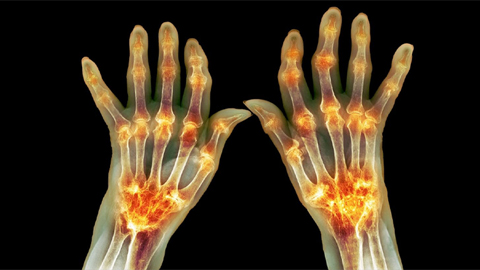
RA patient blood reveals joint innerworkings
Researchers in the Netherlands use mass spectrometry to compare the proteome of plasma and synovial fluid in rheumatoid arthritis patients and find a correlation. Read more about this recent paper in Molecular & Cellular Proteomics.
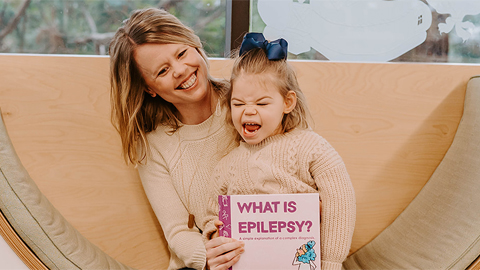
Hope for a cure hangs on research
Amid drastic proposed cuts to biomedical research, rare disease families like Hailey Adkisson’s fight for survival and hope. Without funding, science can’t “catch up” to help the patients who need it most.
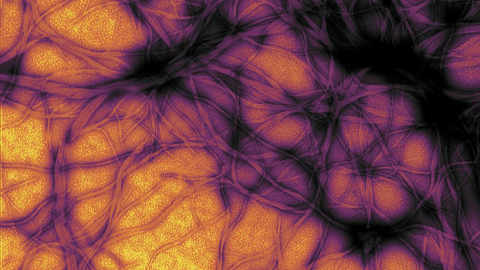
Before we’ve lost what we can’t rebuild: Hope for prion disease
Sonia Vallabh and Eric Minikel, a husband-and-wife team racing to cure prion disease, helped develop ION717, an antisense oligonucleotide treatment now in clinical trials. Their mission is personal — and just getting started.
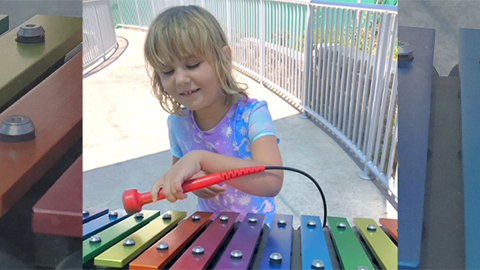
Defeating deletions and duplications
Promising therapeutics for chromosome 15 rare neurodevelopmental disorders, including Angelman syndrome, Dup15q syndrome and Prader–Willi syndrome.
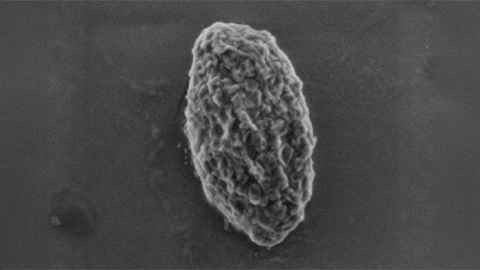
Using 'nature’s mistakes' as a window into Lafora disease
After years of heartbreak, Lafora disease families are fueling glycogen storage research breakthroughs, helping develop therapies that may treat not only Lafora but other related neurological disorders.
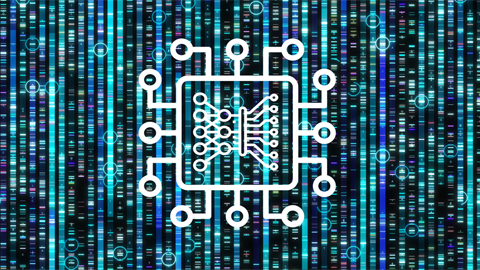
Cracking cancer’s code through functional connections
A machine learning–derived protein cofunction network is transforming how scientists understand and uncover relationships between proteins in cancer.